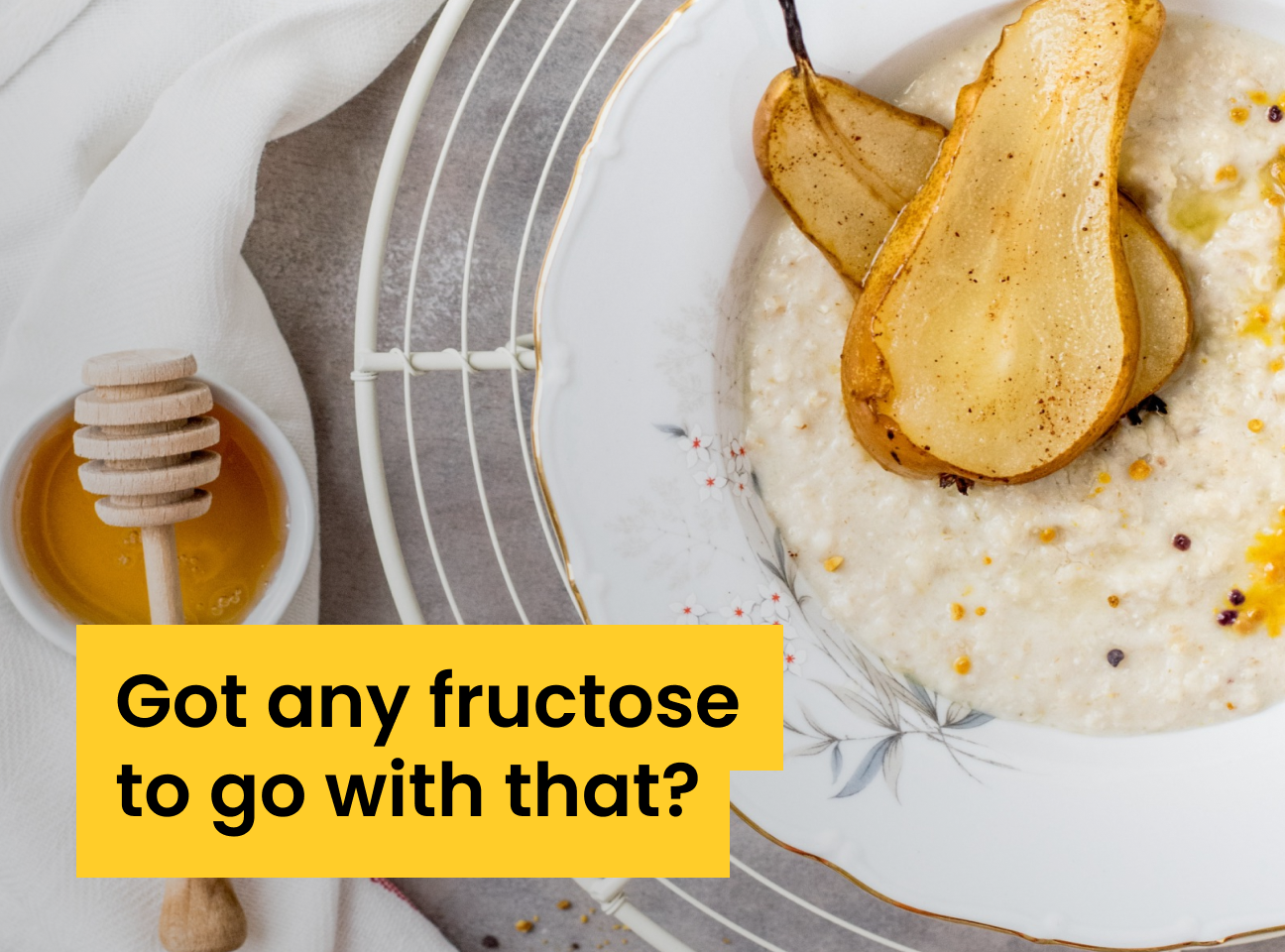
Got any fructose to go with that?
Adding fructose to a cyclist’s pre-race breakfast improves cycling exercise capacity
By Alex Ritson MSc, Head of Education
The study:
Introduction
If you remember your lessons on exercise metabolism, you’ll know that the human body relies primarily on substrates from both muscle and liver glycogen, blood glucose (from liver glycogenolysis and gluconeogenesis) and fatty acids from muscle and lipid stores to fuel endurance exercise. However, when exercise intensities surpass 65% VO₂ max, only carbohydrate (CHO) oxidation keeps pace with the resynthesis demands of ATP, with the reliance on lipid metabolism decreasing (van Loon et al., 2001). Compared to the practically limitless supply of stored energy from lipids, the availability of stored CHO (muscle and liver glycogen) for exercise is constrained to ~600g (average whole-body glycogen content), representing only 4% of the body’s fuel resources (Murray & Rosenbloom, 2018).
Ever since the relationship between increased pre-exercise muscle glycogen concentrations and improved exercise capacity was identified in the late 1960s (Bergström et al., 1967), scientists have continued in their attempts to outsmart the physiological bottleneck of having a limited storage supply of CHO during endurance exercise. Following the initial “classic model” of CHO loading, which involved depleting muscle glycogen stores through exhaustive exercise, followed by three days of CHO restriction and three days of a very high CHO diet (Bergstrom & Hultman, 1966), several studies have uncovered more practical CHO loading regimens that aim to “super compensate” muscle glycogen stores before exercise (Bussau et al., 2002; Sherman et al., 1981). The current consensus, if super compensation of muscle glycogen stores is the objective, is to consume CHO at 10 to 12 g/kg⁻¹ body mass (BM) per day for 36 to 48 h before competition (Thomas et al., 2016).
Compared to research on muscle glycogen, there have been considerably fewer investigations on optimising liver glycogen availability before exercise, despite previous research demonstrating a relationship between increased liver glycogen concentrations and enhanced endurance exercise capacity (Casey et al., 2000). Furthermore, research in rodents that have been genetically modified to upregulate their capacity for liver glycogen storage demonstrate increased exercise capacity, providing further evidence for an association between liver glycogen concentrations and exercise tolerance (López-Soldado et al., 2021).
The absence of research is more likely due to difficulties acquiring liver glycogen samples from research participants (Gonzalez et al., 2016) than to its importance to endurance performance. Indeed, liver glycogen stores serve as a vital reservoir for glucose to maintain blood glucose stability during exercise for the muscle and brain, with its availability linked to both central and peripheral fatigue. In some cases, pre-exercise CHO intake is of greater importance to the liver than muscle glycogen stores, especially before morning endurance exercise, as liver glycogen concentrations fall by ~25% overnight. In contrast, muscle glycogen concentrations after an overnight fast remain virtually unchanged (Iwayama et al., 2021).
These findings, as well as evidence that athletes train harder (Costill et al., 1988; Simonsen et al., 1991) or maintain better exercise performance (Achten et al., 2004; Halson et al., 2004; Simonsen et al., 1991) when carbohydrate intake is better matched to the metabolic demands of exercise, have led to the well-established recommendation that athletes should prioritise 1 to 4 g/kg⁻¹ BM of CHO in the 1 to 4 hours before intense training and/or competition (Thomas et al., 2016). However, knowledge of the composition of dietary CHOs to enhance whole-body CHO availability rather than just muscle glycogen storage remains limited.
In contrast to glucose consumption, which is metabolised throughout the body upon absorption, fructose is predominantly metabolised in the liver, leading to liver glycogen replenishment. Recent research has shown that the co-ingestion of fructose and glucose after strenuous exercise increases liver glycogen replenishment relative to a glucose-only intervention without affecting muscle glycogen replenishment, enhancing total glycogen storage throughout the body. Moreover, this dietary strategy has been shown to further increase CHO availability (Podlogar & Wallis, 2020) and improve endurance exercise capacity (Maunder et al., 2018). Notably, these feeding studies took place after exercise rather than in the morning after an overnight fast. No research had examined the effect of adding fructose to a pre-exercise breakfast meal on exercise performance. Therefore, the purpose of this study was to investigate the hypothesis that combining fructose with a glucose- based CHO breakfast, as compared to consuming solely a glucose-based CHO meal, enhances endurance exercise capacity following an overnight fast in trained cyclists.
Let’s take a look.
Methods
This was a crossover, randomised, double-blind design. Included in the study were eight trained (VO₂ peak of 62.1 ± 5.4 ml/kg⁻¹ min⁻¹) male cyclists following normal sports nutrition practises. Both familiarisation and the two experimental trials involved multiple laboratory visits. The initial visit occurred the afternoon before the exercise capacity test, and participants were instructed to maintain a consistent food and activity diary for both experimental trials.
The initial exercise bout, which took place the afternoon before the exercise capacity test, consisted of 135 minutes of intense interval cycling to deplete glycogen stores. Following the session, participants consumed 30g of a whey protein and 50g of carbohydrate (malto:fructo) beverage, followed by three carbohydrate-rich meals (1.2, 1.2, and 1.5 g/kg/BM⁻¹). The following morning, participants entered the laboratory after an overnight fast and were served a breakfast of 2g/kg/BM⁻¹ of carbohydrates of either rice mixed with a carbohydrate powder (dextrose or fructose) with a 2:1 ratio (rice: powder). Two hours after eating, the participants began cycling at an intensity matching their ventilatory threshold (when CO² output and ventilation increase disproportionately to VO₂ — the earliest signs of disturbance to cellular homeostasis and fatigue).
Results
As a reminder, the investigators intended to test the hypothesis that combining fructose with a glucose-based CHOs breakfast compared to ingesting a glucose-based breakfast leads to enhanced endurance exercise capacity following an overnight fast. As depicted in Figure 2, the results supported the hypothesis that exercise capacity was improved in the FRUC + RICE condition (137.0 ± 22.7 min) than in the GLUC + RICE condition (130.06 ± 19.87 min; p =.046). In addition, the data revealed that neither blood glucose, blood lactate, carbohydrate and fat oxidation rates differed statistically between trials.
Figure 2. Time to task failure during cycling following a breakfast containing GLU + RICE or FRU = RICE. Bars represents mean; circles and connecting lines represents individual participants (n=8). GLU + RICE = rice with added glucose; FRU + RICE = rice with added fructose.
Summary
The primary objective of this research was to determine whether consuming fructose and glucose prior to exercise, as opposed to glucose alone, improves endurance exercise capacity following an overnight fast. The acquired findings confirmed this hypothesis; nonetheless, there were no detectable differences in whole-body substrate metabolism and physiological responses, leaving open the question of the processes underlying the enhanced endurance exercise tolerance.
As liver glycogen stores regulate blood glucose levels, it would be reasonable to assume that the lower blood glucose levels in the glucose-only condition (considering they likely started with less liver glycogen) would have led to the improved exercise performance; yet, when looking at the data, there was no difference in blood glucose levels between the two conditions.
With that said, it’s important to remember, that the regulation of blood glucose can occur via gluconeogenesis (transforming non-carbohydrate substrates such as lactate into glucose), as well as liver glycogenolysis (the breakdown of glycogen into glucose). Thus, the researchers consider the possibility that there are other mechanisms within the body that may detect liver glycogen levels or the rate of breakdown beyond blood glucose levels, causing feedback to the brain and thus causing an earlier onset of fatigue in the GLU + RICE condition. In fact, recent research on rodents provides evidence for this theory (López-Soldado et al., 2017), but, without measurements of liver glycogen concentrations at the beginning and end of the exercise bout — which were not performed and highlights a limitation of the study — this theory remains speculative.
Another important finding of the study was the increase CHO availability as measured by an increase in total CHO oxidation in the FRU + RICE condition compared to the GLU + RICE condition. This was likely a result of higher pre-exercise liver glycogen concentrations in the FRU + RICE condition. In practical terms, when CHO oxidation rates during an endurance event are comparable between two athletes, the athlete with the greater CHO availability will delay the depletion of liver glycogen stores and thus maintain euglycemia and exercise capacity. This example, however, only applies if neither athlete is consuming CHO during the contest.
As illustrated by the Paper-2-Podium grading rubric, the study is limited by the absence of direct measurements of metabolism (direct measures of muscle and liver glycogen storage), and the exercise test. The exercise capacity test has been criticised for not simulating the race strategies used by endurance athletes in competition, which involve undulating levels of effort and a desire to complete the race as fast as humanly possible. However, the authors make the valid point that maintaining a set pace by a group leader, of which an athlete is a part, often determines the outcomes of many endurance events; hence, exercise capacity tests have ecological value in some circumstances.
Paper-to-Podium Matrix Analysis
Next steps
As stated, this study was conducted with participants who did not consume supplementary CHO during exercise. As the reader is aware, the intake of CHO during exercise is routine practice for many endurance athletes as it helps maintain blood glucose levels, decreases liver glycogen output, maintains carbohydrate oxidation, and improves muscular energy balance, thus delaying fatigue and enhancing exercise performance (Hargreaves & Spriet, 2020; Stellingwerff & Cox, 2014). Future research should examine whether there are any performance differences between a fructose-glucose and a glucose-only pre-exercise meal when athletes adhere to race-like sports nutrition dietary recommendations.
Science to practice
Consider an endurance athlete preparing for an endurance race in several weeks, who typically consumes a carbohydrate-rich breakfast with only small amounts of fructose (e.g., a bowl of porridge oats with chopped banana) as their breakfast meal. Given the findings of this study, the practitioner may want to experiment with increasing the amount of fructose (e.g., pears, honey, dates, fruit juice) in the breakfast at a 1:2 ratio to other carbohydrates (see Figure 3) in the meal to increase liver glycogen stores and CHO availability prior to the exercise bout. Alternatively, using a fructose powdered supplement alongside their regular high CHO breakfast meal would be an arguably easier way to ensure fructose quantities reach a 1:2 ratio with other CHO ingested at breakfast (see Figure 4). Considering this, the practitioner must ensure the supplement has been tested for banned substances by a recognised third-party organisation.
FAQ
Shouldn’t we be avoiding fructose consumption for health?
The question of whether fructose is harmful to metabolic health remains hotly debated among researchers. Some researchers assert that eating fructose should be avoided entirely due to its uniquely damaging effects on metabolic health (Lustig et al., 2012), while others have documented that certain populations can consume vast quantities of the monosaccharide without experiencing any metabolic abnormalities (Pontzer et al., 2018). However, the truth, like most topics with opposing viewpoints, generally lies somewhere in the middle. The controversy around fructose stems from the growth in obesity and accompanying metabolic syndrome that occurred from the 1980s onwards, which coincided with an increase in free sugar consumption (e.g., glucose and fructose); although it’s important to note that total calorie intake (~456 kcals more in 2010 than in the 1970s) (DeSilver 2016), and a reduction in physical activity (Church et al., 2011) also occurred during this timeframe. In Europe, two-thirds of fructose intake comes from sucrose (table sugar), and the remaining third is taken in as free fructose. Even if eaten in its free form, fructose is usually accompanied by glucose.
When consumed, fructose is taken in by the cells lining the intestine and enters the bloodstream via GLUT5, a transporter that operates independently of insulin. This fructose, along with glucose, then reaches the liver, where it is absorbed by another insulin-independent transporter called GLUT2. As illustrated in Figure 5, fructose undergoes a transformation in the liver. The enzyme fructokinase first phosphorylates fructose to become fructose 1-phosphate, a process that utilises an ATP molecule. Subsequently, the enzyme aldolase B splits fructose 1-phosphate into two distinct molecules: dihydroxyacetone phosphate (DHAP) and glyceraldehyde. DHAP and glyceraldehyde molecule must then undergo a further transformation by the enzymes triose phosphate isomerase and triose kinase, respectively, to form glyceraldehyde-3-phosphate. On the other hand, glucose is initially transformed into fructose-1,6-biphosphate by the action of the enzyme phosphofructokinase-1 (PFK-1). The metabolic pathways for both glucose and fructose result in the production of dihydroxyacetone phosphate and glyceraldehyde-3-phosphate. These compounds act as intermediaries in the process of glycolysis, eventually leading to the production of pyruvate. An important distinction between glucose and fructose metabolism in the liver lies in the role of PFK-1. This enzyme, which plays a crucial role in the breakdown of glucose, is absent from the pathway of fructose metabolism. PFK-1 is a highly regulated enzyme. It gets activated in conditions where energy is depleted (for instance, in the presence of AMP or fructose-2,6-bisphosphate) and is inhibited when there is an energy surplus (for example, in the presence of ample ATP, citrate, or hydrogen ions). This lack of regulation in fructose metabolism means there’s no feedback mechanism in place to slow or stop the process when needed. In some circumstances, this can lead to unchecked accumulation of acetyl-CoA, a molecule produced when fructose undergoes glycolysis. When the production of acetyl-CoA surpasses the rate at which the Krebs cycle can use it, the excess acetyl-CoA triggers a process known as De Novo Lipogenesis (DNL), leading to the creation of new fat molecules. Over time, this can result in the accumulation of fat in the liver.
Figure 5. The metabolism of glucose and fructose in the liver
As an analogy, imagine a factory, which symbolises the liver, where workers — who represent enzymes — handle two types of raw materials: fructose and glucose. Both materials are used to produce a common end product: energy, represented by ATP. When glucose arrives, it behaves like a diligent employee who strictly adheres to the factory’s regulations. The glucose processing line includes a supervisor, represented by the enzyme PFK-1. This supervisor assesses the factory’s current energy supply before initiating production. If the factory already has an ample supply of energy, the supervisor regulates the pace of the processing line, slowing or even stopping it from preventing unnecessary production. On the other hand, fructose behaves more like a renegade worker, disregarding the factory’s protocols. The fructose processing line lacks a supervisor akin to PFK-1. Consequently, this line operates continuously at maximum speed without any consideration for the factory’s existing energy supply. This unchecked operation often leads to an excess of energy, which is then converted and stored as an alternative product: fat, via DNL.
While excessive fructose consumption is associated with weight gain and increased liver fat, research suggests that these metabolic abnormalities occur largely under conditions of calorie excess (Stricker et al., 2021). Current meta-analyses of randomised controlled trials demonstrate no metabolic effects with isocaloric (when Calories are matched between conditions, e.g., high-fructose and low-fructose conditions) fructose consumption when energy balance remains unchanged (Stricker et al., 2021). It has been proposed that the rise in liver fat accumulation (lipogenesis) occurs when the liver glycogen stores are fully saturated, as would be assumed in conditions of calorie excess (Hengist et al., 2019). In contrast, if liver glycogen stores are depleting faster than liver glycogen synthesis, regardless of fructose intake, this should reduce DNL and liver lipogenesis. This research and the liver glycogen storage theory align with previous findings on endurance athlete’s consuming ~460g of supplemental carbohydrates (sugars) daily in the Tour de France yet presented no metabolic abnormalities (Saris et al., 1989). Similarly, hunter-gatherer populations have been reported to consume up to ~15% (ranging monthly between 1-50%) of their total Calories from honey (~35g fructose/100g honey) and bee larvae (Pontzer et al., 2018) — although these reports are likely to be underestimations — and exhibit excellent metabolic and cardiovascular health. Conversely, in non-exercising individuals, high intakes of fructose lead to metabolic problems and high rates of DNL within days (Acheson et al., 1988).
Thus, research suggests that fructose ingestion has no negative metabolic effects under energy balance or hypocaloric conditions. Furthermore, DNL and consequent liver lipogenesis are reduced when liver glycogen levels are not fully saturated. Taken together, the metabolic health implications of high fructose consumption are unlikely to be relevant to endurance athletes with high exercise energy expenditures and training volumes that likely deplete liver glycogen stores repeatedly throughout their weekly training, in addition to remaining in prolonged states of negative energy balance.
Take home
In trained male cyclists, the addition of fructose to a CHO-rich breakfast (2 g/kg⁻¹ BM of CHO) improved exercise capacity compared to the addition of glucose. The mechanism for the improved performance remains unknown, and further research needs to discern whether such performance benefits would be sustained when athletes consume supplemental CHO during the exercise bout. This study does imply, however, that pre-exercise carbohydrate intakes that target both the liver and the muscle are likely ideal for optimising CHO availability and performance before competition or intense training sessions.
References
- Acheson, K. J., Schutz, Y., Bessard, T. M., Anantharaman, K., Flatt, J.-P. P., & Jequier, E. M. (1988). Glycogen storage capacity and de novo lipogenesis massive carbohydrate overfeeding in man. American Journal of Clinical Nutrition, 48(2), 240–247. https://doi.org/10.1080/07315724.2014.911668
- Achten, J., Halson, S. L., Moseley, L., Rayson, M. P., Casey, A., & Jeukendrup, A. E. (2004). Higher dietary carbohydrate content during intensified running training results in better maintenance of performance and mood state. Journal of Applied Physiology, 96(4), 1331–1340. https://doi.org/10.1152/japplphysiol.00973.2003
- Casey, A., Mann, R., Banister, K., Fox, J., Morris, P. G., Macdonald, I. A., Greenhaff, P. L., & Green, P. L. (2000). Effect of carbohydrate ingestion on glycogen resynthesis in human liver and skeletal muscle, measured by 13 C MRS. http://www.ajpendo.org
- Church, T. S., Thomas, D. M., Tudor-Locke, C., Katzmarzyk, P. T., Earnest, C. P., Rodarte, R. Q., Martin, C. K., Blair, S. N., & Bouchard, C. (2011). Trends over 5 decades in U.S. occupation-related physical activity and their associations with obesity. PLoS ONE, 6(5), 1–7. https://doi.org/10.1371/journal.pone.0019657
- Close, G. L., Kasper, A. M., & Morton, J. P. (2019). From Paper to Podium: Quantifying the Translational Potential of Performance Nutrition Research. Sports Medicine. https://doi.org/10.1007/s40279-018-1005-2
- Costill, D., Flynn, M., Kirwan, J., Houmard, J., Mitchell, J., Thomas, R., & Park, S. (1988). Effects of Intensified Training on Glycogen and Swimming Performance. In Medicine and science in sports and exercise (Vol. 20, Issue 3, pp. 249–254). Gonzalez, J. T., Fuchs, C. J., Betts, J. A., & van Loon, L. J. C. (2016). Liver glycogen metabolism during and after prolonged endurance-type exercise. American Journal of Physiology – Endocrinology and Metabolism, 311(3), E543–E553. https://doi.org/10.1152/ajpendo.00232.2016
- Halson, S. L., Lancaster, G. I., Achten, J., Gleeson, M., & Jeukendrup, A. E. (2004). Effects of carbohydrate supplementation on performance and carbohydrate oxidation after intensified cycling training. Journal of Applied Physiology, 97(4), 1245–1253. https://doi.org/10.1152/japplphysiol.01368.2003
- Hargreaves, M., & Spriet, L. L. (2020). Skeletal muscle energy metabolism during exercise. Nature Metabolism. https://doi.org/10.1038/s42255-020-0251-4
- Hengist, A., Koumanov, F., & Gonzalez, J. T. (2019). Fructose and metabolic health: governed by hepatic glycogen status? The Journal of Physiology, JP277767. https://doi.org/10.1113/JP277767
- Iwayama, K., Tanabe, Y., Tanji, F., Ohnishi, T., & Takahashi, H. (2021). Diurnal variations in muscle and liver glycogen differ depending on the timing of exercise. Journal of Physiological Sciences, 71(1). https://doi.org/10.1186/s12576-021-00821-1 Jeukendrup, A. E., Craig, N. P., & Hawley, J. A. (2000). The bioenergetics of world class cycling. Journal of Science and Medicine in Sport, 3(4), 414–433. https://doi.org/10.1016/S1440-2440(00)80008-0
- López-Soldado, I., Fuentes-Romero, R., Duran, J., & Guinovart, J. J. (2017). Effects of hepatic glycogen on food intake and glucose homeostasis are mediated by the vagus nerve in mice. Diabetologia, 60(6), 1076–1083. https://doi.
- org/10.1007/s00125-017-4240-4
- López-Soldado, I., Guinovart, J. J., & Duran, J. (2021). Increased liver glycogen levels enhance exercise capacity in mice. Journal of Biological Chemistry, 297(2). https://doi.org/10.1016/j.jbc.2021.100976
- Lustig, R. H., Schmidt, L. A., & Brindis, C. D. (2012). The toxic truth about sugar. Nature, 27–29.
- Maunder, E., Podlogar, T., & Wallis, G. A. (2018). Postexercise Fructose-Maltodextrin Ingestion Enhances Subsequent Endurance Capacity. Medicine and Science in Sports and Exercise, 50(5), 1039–1045. https://doi.org/10.1249/ MSS.0000000000001516
- Podlogar, T., & Wallis, G. A. (2020). Impact of Post-Exercise Fructose-Maltodextrin Ingestion on Subsequent Endurance Performance. Frontiers in Nutrition, 7(82).
- Pontzer, H., Wood, B. M., & Raichlen, D. A. (2018). Hunter-gatherers as models in public health. In Obesity Reviews (Vol. 19, pp. 24–35). Blackwell Publishing Ltd. https://doi.org/10.1111/obr.12785
- Saris, W. H. M., van Erp-Baart, M. A., Brouns, F., Westerterp, K. R., & ten Hoor, F. (1989). Study on food intake and energy expenditure during extreme sustained exercise: The Tour de France. International Journal of Sports Medicine, 10(SUPPL. 1). https://doi.org/10.1055/s-2007-1024951
- Simonsen, J. C., Sherman, W. M., Lamb, D. R., Dernbach, A. R., Doyle, J. A., & Strauss, R. (1991). Dietary carbohydrate, muscle glycogen, and power output during rowing training. Journal of Applied Physiology, 70(4), 1500–1505. https:// doi.org/10.1152/jappl.1991.70.4.1500
- Stellingwerff, T., & Cox, G. R. (2014). Systematic review: Carbohydrate supplementation on exercise performance or capacity of varying durations. Applied Physiology, Nutrition, and Metabolism, 39(9), 998–1011. https://doi.org/10.1139/ apnm-2014-0027
- Stricker, S., Rudloff, S., Geier, A., Steveling, A., Roeb, E., & Zimmer, K. P. (2021). Fructose Consumption—Free Sugars and Their Health Effects. Deutsches Arzteblatt International, 118(5), 71–80. https://doi.org/10.3238/arztebl.m2021.0010
- Thomas, D. T., Erdman, K. A., & Burke, L. M. (2016). American College of Sports Medicine Joint Position Statement. Nutrition and Athletic Performance. Medicine and Science in Sports and Exercise, 48(3), 543–568. https://doi.org/10.1249/MSS.0000000000000852